Andreas Nunnenkamp
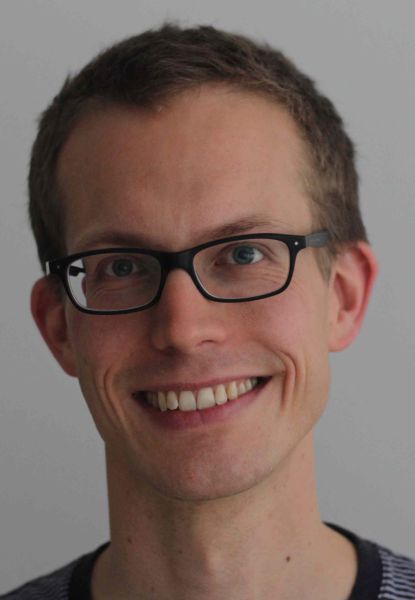
Dr Andreas Nunnenkamp
Royal Society University Research Fellow
Office: 984 Rutherford Bld
Phone: +44(0)1223 3 37479
Email: an448 @ cam.ac.uk
Google Scholar
ResearcherID
TCM Group, Cavendish Laboratory
19 JJ Thomson Avenue,
Cambridge, CB3 0HE UK.
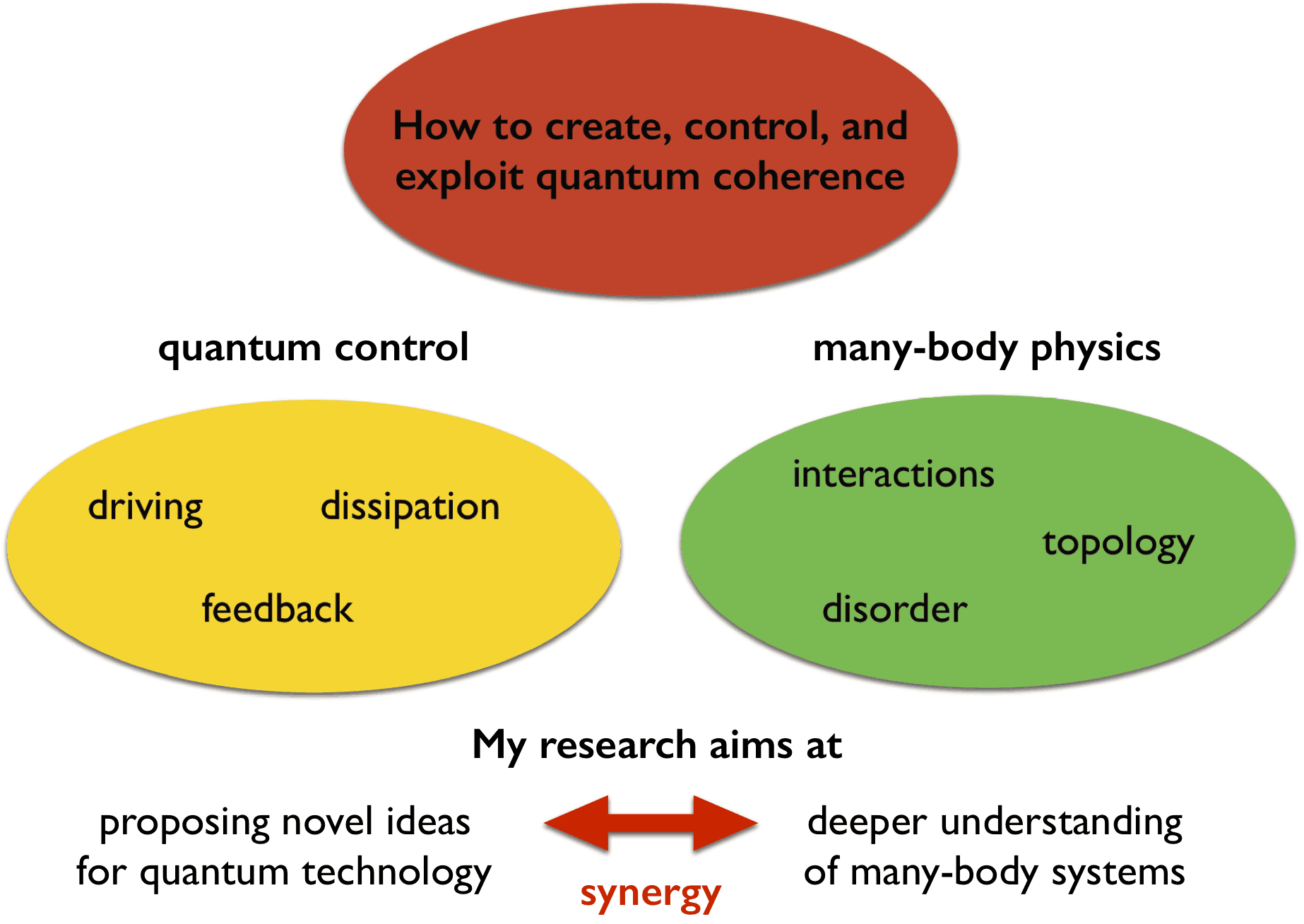
Research
A central theme of my research is quantum coherence in atomic, mesoscopic, and optical systems and, in particular, how to exploit it for fundamental table-top science and future quantum technologies. This puts my work at the interface of theoretical quantum optics, atomic and laser physics, condensed matter theory, and quantum information science. In particular, I have worked on strong correlations, quantum-enabled technology, and control of ultracold atomic gases, superconducting circuits, and cavity optomechanical systems.
Cavity optomechanics is a rapidly-growing field in which mechanical degrees of freedom are coupled to modes of the electromagnetic field inside optical or microwave resonators. Adapting laser-cooling techniques from atomic physics several experiments have recently observed mechanical motion close to the quantum ground-state. This paves the way to exploit these systems for the engineering of phonon and photons at the nanoscale - with exciting applications for quantum science and technology.
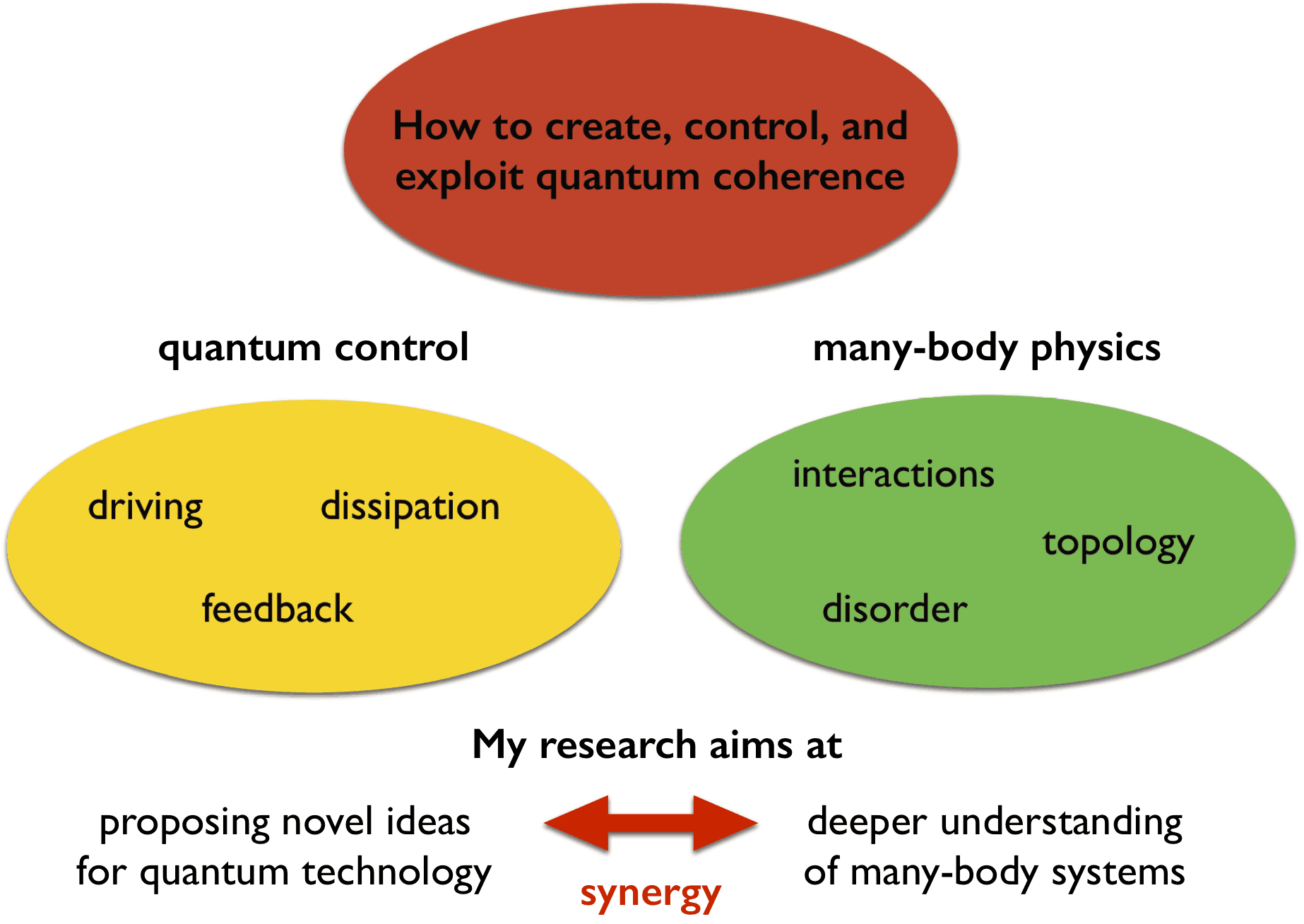
In Plain English
Light is an electromagnetic wave carrying energy, but also momentum. That means, if light is reflected off a surface, this surface will experience a mechanical force, the radiation-pressure force. In everyday circumstances, this force is typically negligible: we do not notice the sun light reflected off our skin. If, however, light is trapped between two almost perfectly reflecting mirrors (so it bounces back and forth many times) and one mirror is light enough (so it responds to even a tiny force), the light has a strong impact on its motion. This dependence of light and mechanical motion is at the heart of cavity optomechanical systems.
This paradigm has been implemented in systems whose frequency and mass span many orders of magnitude. The largest setups in this group are the kilogram-heavy mirrors of the LIGO interferometer for gravitational-wave detection. At much smaller scales, there are the micro- to nano-meter cantilevers, membranes, and wires in the Kilohertz to Megahertz regime, but also surprisingly different architectures with similar physics. One example are microwave circuits made of superconducting wires where at least one of the capacitors is mechanically compliant. This means, the top plate of a parallel plate capacitor is able to mechanically move and modulate the resonance frequency of the circuit. Finally, there are setups with even more microscopic mechanical elements, e.g. the collective motion of a few thousand ultracold atoms in an optical resonator - a truly microscopic variant of this idea.
Over the last decade, there has been exciting progress in fabricating mechanical structures with better and better mechanical and optical properties. Today, these devices are so weakly damped that they oscillate more than a million times before loosing half of their energy. With these high-quality mechanical oscillators, several radiation-pressure effects have been demonstrated. Perhaps most impressively, experimentalists have cooled these devices to the quantum regime adapting laser-cooling methods from atomic physics. That means, the thermal center-of-mass motion of these small, but macroscopic objects is now close to 'absolute zero'. This is an extremely interesting result in itself, but it is also an ideal starting point for applications. As devices suffer from thermal noise, lower temperatures mean less noise, more sensitivity, and longer coherence times of the devices.
Featured Publications
- Topological framework for directional amplification in driven-dissipative cavity arrays. Nature Communications 11 3149 (2020)
- Period-n Discrete Time Crystals and Quasicrystals with Ultracold Bosons. Phys. Rev. Lett. 123 150601 (2019)
- Topological magnon amplification. Nature Communications 10 3937 (2019)
- Conditional Dynamics of Optomechanical Two-Tone Backaction-Evading Measurements. Phys. Rev. Lett. 123 093602 (2019)
- Dissipation-Induced Instabilities of a Spinor Bose-Einstein Condensate Inside an Optical Cavity. Phys. Rev. Lett. 122 193605 (2019)
- Optical backaction-evading measurement of a mechanical oscillator. Nature Communications 10 2086 (2019)
- Slow Growth of Out-of-Time-Order Correlators and Entanglement Entropy in Integrable Disordered Systems. Phys. Rev. Lett. 122 020603 (2019)
- Quantum-limited directional amplifiers with optomechanics. Phys. Rev. Lett. 120 023601 (2018)
- Robustness of Majorana edge modes and topological order -- exact results for the symmetric interacting Kitaev chain with disorder. Phys. Rev. B 96 241113(R) (2017)
- Nonreciprocal reconfigurable microwave optomechanical circuit. Nature Communications 8 604 (2017)
- Quantum synchronization blockade: Quantization hinders synchronization of identical oscillators. Phys. Rev. Lett. 118 243602 (2017)
- Engineered dissipative reservoir for microwave light using circuit optomechanics. Nature Physics 13 787 (2017)
- Appearance and disappearance of quantum correlations in measurement-based feedback control of a mechanical oscillator. Phys. Rev.X 7 011001 (2017)
- Triple-resonant Brillouin light scattering in magneto-optical cavities. Phys. Rev. Lett. 117 133602 (2016)
- Genuine quantum signatures in synchronization of anharmonic self-oscillators. Phys. Rev. Lett. 117 073601 (2016)
- Strong mechanical driving of a single electron spin. Nature Physics 11 820 (2015)
- Quantum-limited amplification and parametric instability in the reversed dissipation regime of cavity optomechanics. Phys. Rev. Lett. 113 023604 (2014)
- Quantum synchronization of a driven self-sustained oscillator. Phys. Rev. Lett. 112 094102 (2014)
- Signatures of nonlinear cavity optomechanics in the weak coupling regime. Phys. Rev. Lett. 111 053603 (2013)
- Majorana qubit rotations in microwave cavities. Phys. Rev. Lett. 110 107006 (2013)
- Proposal for entangling remote micromechanical oscillators. Phys. Rev. Lett. 107 123601 (2011)
- Single-photon optomechanics. Phys. Rev. Lett. 107 063602 (2011)